The Richtmyer-Meshkov Instability Utilizing the Rayleigh-Taylor Instability for the Initial Condition
A membraneless interface between two gases is created using the
retractable plate technique in the shock tube using the setup in the
diagnostics page. Initially, a
copper plate with an imposed sinusoidal perturbation (Fig. 1)
separates a heavy gas (CO2) above the plate from a lighter gas
(air) beneath the plate. When the plate is retracted
through a slot in the back wall of the shock tube (Fig. 2) the
two fluids begin to mix due to the onset of the
Rayleigh-Taylor (RT) instability. The interface is
visualized with planar imaging utilizing Mie scattering from
smoke particles that are seeded in the lighter gas, thus, the
heavy gas appears black while the lighter gas appears white. |
|
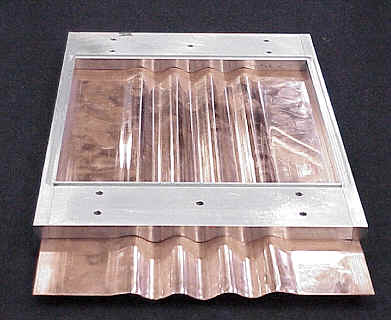
|
|
Fig. 1 Sinusoidal plate. |
|
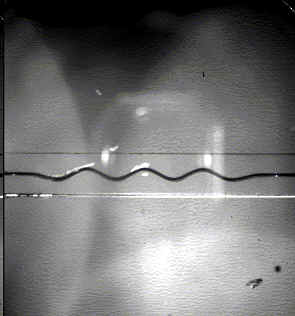
|
|
Fig. 2 Machined slot in the back wall of the shock
tube. |
The RT development is shown in Fig. 3. The initial
perturbation (cosine, h0=3.2
mm, l=38.1 mm) grows in amplitude and remains predominantly sinusoidal
in nature until approximately 110 ms.
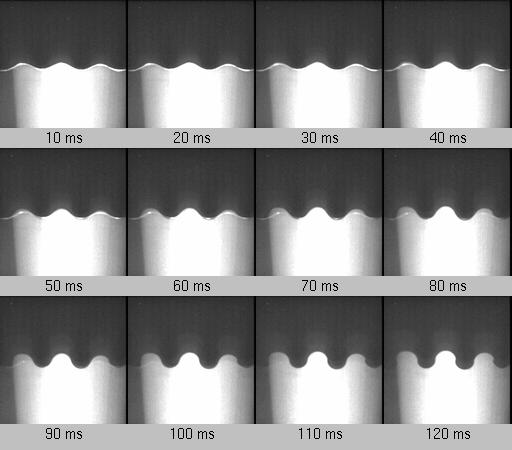 |
|

Evolution of central air mushroom. For the development
of all three wavelengths, click here (2.3 Meg). |
Fig. 3 Evolution of RT instability. CO2
(dark) above air (light). |
|
|
The experimental images are analyzed with an image processing algorithm
to determine the location of the interface. Two full
wavelengths are analyzed and the results are shown in Figs. 4 and
5. Fourier analysis is only possible up to 120 ms because at
this time there are portions of the interface where there are
multiple ordinates for a given abscissa. The dominant modal
growth is the fundamental with the third harmonic being the next
highest at later times.
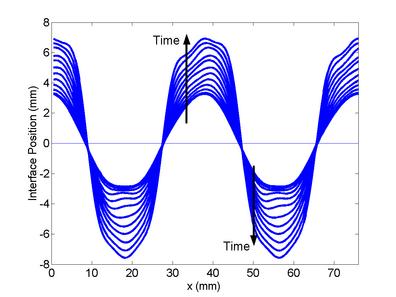 |
|
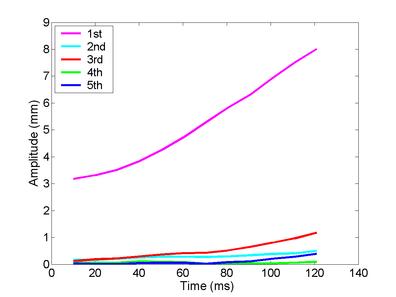 |
Fig. 4 Fourier transformed interface with first five
harmonics |
|
Fig. 5 Spectral amplitudes of the first five
harmonics. |
A strongly shocked (M=3.06) interface is shown in its initial
developmental stages in Fig. 6. Each image was obtained in a
separate experiment with a similar initial condition. In Fig.
6a the edge of the copper sheet is seen reflecting light prior to
being withdrawn through the wall of the shock tube. This type
of interface is referred to as slow-fast (SF) because the shock
travels from the gas with the slower sound speed (CO2)
into the gas with the faster sound speed (air). Typically, the
first gross phenomena seen in a shocked SF interface is the
compression and flattening of the interface which is shown clearly
in Fig. 6b. Then, a phase reversal occurs, and what was
initially a peak becomes a valley that grows in amplitude, and
vice-versa, Figs. 6c-d. This reversal phenomena can be
contrasted to that of a fast-slow interface where a peak is
compressed and then grows in amplitude. The shock wave can be
seen in Figs. 6b-c as the bright line directly beneath the
interface- the initially planar shock wave, which would appear as a
line in two dimensions, has been distorted by refraction through the
SF interface. Later stages of the instability development are
shown in Fig. 7. The amplitudes have grown dramatically in Fig
7a, and after approximately 1 ms of being shocked, the images (Figs.
7b-d) suggest the flow is transitioning to a turbulent mixing layer.
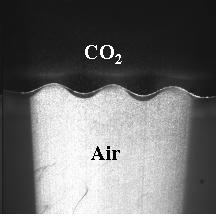 |
|
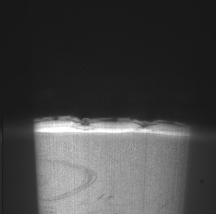 |
|
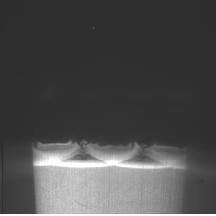 |
|
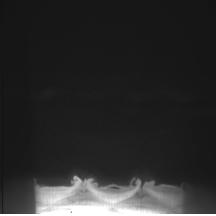 |
(a) t=0 |
|
(b) t=5 ms |
|
(c) t=36 ms |
|
(d) t=39 ms |
Fig. 6 Early stages of the shocked
interface development showing compression and phase reversal |
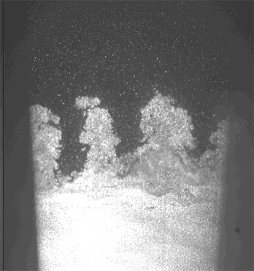 |
|
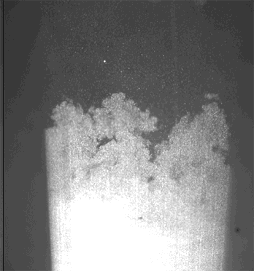 |
|
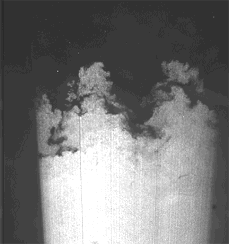 |
|
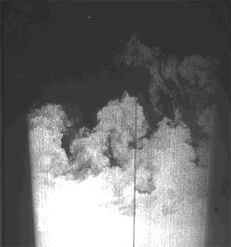 |
(a) t=0.64 ms |
|
(b) t=1.37 ms |
|
(c) t=1.80 ms |
|
(d) t=2.1 ms |
Fig.7 Late stage development of the
instability showing the spikes and bubbles, and turbulent
mixing. |
The peak-to-peak amplitude of the shocked interface is measured
and plotted in Fig. 8. The experimental data is plotted in
green points and is compared to the evaluations of two analytical
models. The images in Fig. 7 show a turbulent mixing region,
however, the bubble and spike nature are still evident, thus, the
data is compared to an analytical model in each class. The
models are evaluated twice, at the low and high values of the
initial data (since the amplitude of the shocked interface varies
from shot to shot, primarily due to the uncertainty of diaphragm
rupture time, +/- 20 ms). The spike-bubble model (SB) shows
less sensitivity to the initial condition and therefore less spread
at later times. The experimental data compares more favorably
with the turbulent-mixing-layer power-law (PL) model over the entire
range of time considered.
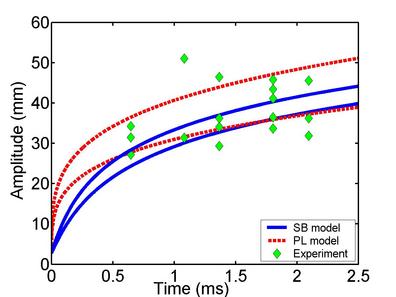
|
Fig. 8 The peak-to-peak amplitude compared
with a spike-bubble (SB) model and a turbulent mixing layer
(PL) model. |
For more details on this work, please see: Puranik, P. B., J. G. Oakley, M. H. Anderson and R. Bonazza,
Experimental study of the
Richtmyer-Meshkov instability induced by a Mach 3 shock wave, Shock Waves, vol. 13, no. 6, pp. 413-429, 2004.
|
|